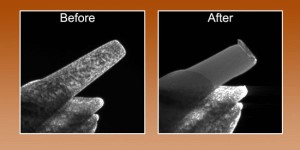
Compression of a nickel pillar whose free end has a
diameter of about 150 nanometers. Before compression (left) the
pillar has a high density of defects, visible as dark mottling.
After compression all the defects have been driven out, a
previously unobserved process known as "mechanical annealing."
|
"What controls the deformation of a metal
object is the way that defects, called dislocations, move along planes
in its crystal structure," Minor says. "The result of dislocation slip
is plastic deformation. For example, bending a paper clip causes its
trillions of dislocations per square centimeter to tangle up and
multiply as they run into one another and slide along numerous slip
planes."
In general, mechanical deformation tends to increase the number of
dislocations in a material. But for small-scale structures, with a
much greater proportion of surface area to volume, the process can be
very different. The videotaped images from the electron microscope
helped the researchers understand why nanoscale nickel pillars are so
strong by allowing them to observe changes in the microstructure of
the pillars during deformation - including a
never-before-seen process the researchers dubbed "mechanical annealing."
(In bulk materials, annealing, a treatment that reduces the density of
defects, is usually accomplished by heating.)
Minor says, "The first thing we observed was that, before the test,
the nanoscale pillars of nickel were full of dislocations. But as we
compressed the pillar, all the dislocations were driven out of the
material - literally reducing the dislocation
density by 15 orders of magnitude and producing a perfect crystal. We
called this effect mechanical annealing."
The pillars Minor and his colleagues tested were machined from pure
nickel using a focused ion beam (FIB), a new technique for small-scale
mechanical-compression testing first described in 2004 by Michael
Uchic of the U.S. Air Force Research Laboratory and his colleagues.
The FIB technique makes it possible to create much smaller structures
than the metal "whiskers" first studied in the 1950s, which are made
by growing crystals.
Some of the dislocations the researchers observed in the machined
pillars were relatively shallow and caused by the ion beams themselves.
Others extended through the crystal and were presumably pre-existing
defects. Under compression, mechanical annealing caused both kinds of
defect to vanish.
"Essentially all the dislocations escape from the crystal at the
surface, and you do not get storage of dislocations like you would in
larger crystals," Minor says. "What results is a process called 'dislocation
starvation,' recently proposed by William D. Nix of Stanford, among
others, which has quickly became one of the leading theories of why
smaller structures are stronger."
Minor explains, "The idea is that if dislocations escape the material
before they can interact and multiply, there are not enough active
dislocations to enable the imposed deformation. The structure can only
deform after new dislocations are created." This is precisely the
process he and his colleagues observed with NCEM's In Situ Microscope,
strong evidence that "dislocation starvation" is the correct
explanation for the increased strength of small structures.
What happens if a defect-free nanoscale nickel pillar continues to be
compressed? Something has to give, which happens when new sources of
dislocation "nucleate" in the material. As the existing dislocations
disappear in the pillar because of mechanical annealing, the
nucleation of new dislocation sources happens at progressively higher
stresses.
In the pillar structures, plastic deformation may take the form of
sudden flattening, bulging, twisting, or shearing of the pillar, as
bursts of new dislocations propagate through it. Or the hardened
pillars, made stronger by mechanical annealing, may punch right down
into the substrate - even though pillar and
substrate are the same continuous piece of metal. Both processes were
captured in the In Situ Microscope's dramatic videotaped experiments.
The FIB machining used by the NCEM researchers produced nickel pillars
that were slightly tapered, and the researchers noted that this
geometry affected where and how plastic deformation occurred,
generally being greater in the smaller-diameter, free end (top) of the
pillar.
In larger pillars, those approaching 300 nanometers in diameter,
mechanical annealing was not complete, and some dislocations remained
visible even after compression. Yet even these pillars exhibited
enhanced strength, and progressively higher stresses were needed to
continue deformation - underlining the point
that it is the creation of mobile defects that determines strength in
these small volumes.
"The beauty of the pillar-testing geometry is that we can
straightforwardly define stress. Then we can correlate the measured
stresses with discrete plastic events recorded in situ and more
clearly interpret the quantitative data from our experiments," says
Minor. "The debate over what determines the strength of a small
structure has come down to almost a chicken-and-egg question � is
something strong because you need a high stress to move a dislocation
that is already there? Or is it strong because you need a high stress
to nucleate a new dislocation? In this case, it seems that source
nucleation - that is, the 'egg'
- is the determining factor."
|